Electronic and Thermoelectric Properties on Rutile SnO2 Under Compressive and Tensile Strains Engineering
Abstract
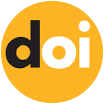
Keywords
References
S. Das and V. Jayaraman, “SnO2: a comprehensive review on structures and gas sensors,” Prog. Mater. Sci., vol. 66, pp. 112–255, Oct. 2014. doi: 10.1016/j.pmatsci.2014.06.003. Crossref
M. B. Sahana, C. Sudakar, A. Dixit, J. S. Thakur, R. Naik, and V. M. Naik, “Quantum confinement effects and bandgap engineering of SnO2 nanocrystals in a MgO matrix,” Acta Mater, vol. 60, no. 3, pp. 1072–1078, Feb. 2012, doi: 10.1016/j.actamat.2011.11.012. Crossref
A. Paulson, M. Sabeer, and P. P. Prayudmnan, “Enhanced thermoelectric property of oxygen deficient nickel doped SnO2 for high temperature application,” Mater. Res. Express, vol. 5, pp. 1–21, Apr. 2018, doi: 10.1088/2053-1591/aabd64. Crossref
S. Yu, L. Li, D. Xu, H. Dong, and Y. Jin, “Characterization of SnO2/Cu/SnO2 multilayers for high performance transparent conducting electrodes,” Thin Solid Films, vol. 562, pp. 501–505, Jul. 2014, doi: 10.1016/j.tsf.2014.04.064. Crossref
Z. Kerrami, A. Sibari, O. Mounkachi, A. Benyoussef, and M. Benaissa, “SnO2 improved thermoelectric properties under compressive strain,” Comput. Condens. Matter, vol. 18, Mar. 2019, Art. no. e00356, doi: 10.1016/j.cocom.2018.e00356. Crossref
A. J. Minnich, M. S. Dresselhaus, Z. F. Ren, and G. Chen, “Bulk nanostructured thermoelectric materials: current research and future prospects,” Energy Environ. Sci., vol. 2, no. 5, pp. 466–479, Feb. 2009, doi: 10.1039/b822664b. Crossref
G. S. Nolas, J. Sharp, and J. Goldsmid, Thermoelectrics: basic principles and new materials developments, 1st ed., Heidelberg, Germany: Springer Berlin, 2013, doi: 10.1007/978-3-662-04569-5. Crossref
A. R. Nugraha, N. L. Kartika, Dedi, and A. A. Nugroho, “Thermoelectric properties of SnO2/Bi2Te3 composite,” Mater. Sci. Forum, vol. 1028, pp. 99–104, Apr. 2021, doi: 10.4028/www.scientific.net/MSF.1028.99. Crossref
S. Fayette, D. S. Smith, A. Smith, and C. Martin, “Influence of grain size on the thermal conductivity of tin oxide ceramics,” J. Eur. Ceram. Soc., vol. 20, no. 3, pp. 297–302, Mar. 2000, doi: 10.1016/S0955-2219(99)00171-5. Crossref
M. M. Bagheri-Mohagheghi, N. Shahtahmasebi, M. R. Alinejad, A. Youssefi, and M. Shokooh-Saremxi, “Fe-doped SnO2 transparent semi-conducting thin films deposited by spray pyrolysis technique: thermoelectric and p-type conductivity properties,” Solid State Sci., vol. 11, no. 1, pp. 233–239, Jan. 2009, doi: 10.1016/j.solidstatesciences.2008.05.005. Crossref
K. Rubenis, S. Populoh, P. Thiel, S. Yoon, U. Müller, and J. Locs, “Thermoelectric properties of dense Sb-doped SnO2 ceramics,” J. Alloys Compd., vol. 692, pp. 515–521, Jan. 2017, doi: 10.1016/j.jallcom.2016.09.062. Crossref
N. L. Kartika et al., “Thermopower enhancement of rutile-type SnO2 nanocrystalline using facile co-precipitation method,” J. Elektr. Telekom., vol. 20, no. 2, pp. 82–88 , Dec. 2020, doi: 10.14203/jet.v20.82-88. Crossref
F. Delorme et al., “Nanostructuring of dense SnO2 ceramics by spark plasma sintering,” Ceram. Int., vol. 45, no. 7, pp. 8313–8318, May 2019, doi: 10.1016/j.ceramint.2019.01.138. Crossref
N. Kumar, G. K. Sidhu, and R. Kumar, “Correlation of synthesis parameters to the phase segregation and lattice strain in tungsten oxide nanoparticles,” Mater. Res. Express, vol. 6, no. 7, Apr. 2019, Art. no. 0750 doi: 10.1088/2053-1591/ab12a5. Crossref
W. Zhou, Y. Liu, Y. Yang, and P. Wu, “Bandgap engineering of SnO2 by epitaxial strain: experimental and theoretical investigations,” J. Phys. Chem. C., vol. 118, no. 12, pp. 6448–6453, Mar. 2014, doi: 10.1021/jp500546r. Crossref
H. Absike, M. Hajji, H. Labrim, B. Hartiti, and H. Ez-Zahraouy, “Strain effects on the electronic and thermoelectric properties of copper oxide,” Comput. Condens. Matter, vol. 16, Sep. 2018, Art. no. e00322 doi: 10.1016/j.cocom.2018.e00322. Crossref
W. Kohn and L. J. Sham, “Self-consistent equations including exchange and correlation effects,” Phys. Rev., Nov. 1965, Art. no. A1133, doi: 10.1103/PhysRev.140.A1133. Crossref
J. P. Perdew et al., “Restoring the density-gradient expansion for exchange in solids and surfaces,” Phys. Rev. Lett., vol. 100, no. 13, Apr. 2008, Art. no. 136406, doi: 10.1103/PhysRevLett.100.136406. Crossref
J. W. Song, K. Yamashita, and K. Hirao, “Communication: a new hybrid exchange correlation functional for bandgap calculations using a short-range Gaussian attenuation (Gaussian-Perdue-Burke- Ernzerhof),” J. Chem. Phys., vol. 135, no. 7, Aug. 2011, Art. no. 071103, doi: 10.1063/1.3628522. Crossref
C. Adamo and V. Barone, “Toward reliable density functional methods without adjustable parameters: the PBE0 model,” J. Chem. Phys., vol. 110, no. 13, pp. 6158–6170, Mar. 1999, doi: 10.1063/1.478522. Crossref
J. Heyd, G. E. Scuseria, and M. Ernzerhof, “Hybrid functionals based on a screened coulomb potential,” J. Chem. Phys., vol. 118, no. 18, pp. 8207–8215, May 2003, doi: 10.1063/1.1564060. Crossref
P. Giannozzi et al., “Quantum espresso: a modular and open-source software project for quantum simulations of materials,” J. Phys. Condens. Matter, vol. 21, no. 39, Jun. 2009, Art. no. 395502, doi: 10.1088/0953-8984/21/39/395502. Crossref
P. E. Blöchl, “Projector augmented-wave method,” Phys. Rev. B, vol. 50, no. 24, pp. 17953–17979, Dec. 1994, doi: 10.1103/PhysRevB.50.17953. Crossref
A. A. Mostofi et al., “An updated version of Wannier90: a tool for obtaining maximally-localized Wannier functions,” Comput. Phys. Commun., vol. 185, no. 8, pp. 2309–2310, Aug. 2014, doi: 10.1016/j.cpc.2014.05.003. Crossref
G. Pizzi, D. Volja, B. Kozinsky, M. Fornari, and N. Marzari, “BoltzWann: a code for the evaluation of thermoelectric and electronic transport properties with a maximally-localized Wannier functions basis,” Comput. Phys. Commun., vol. 185, pp. 422–429, May 2013, doi: 10.1016/j.cpc.2013.09.015. Crossref
K. B. Spooner, A. M. Ganose, and D. O. Scanlon, “Assessing the limitations of transparent conducting oxides as thermoelectrics,” J. Mater. Chem. A, vol. 8, no. 24, pp. 11948–11957, Jun. 2020, doi: 10.1039/d0ta02247k. Crossref
D. O. Scanlon and G. W. Watson, “On the possibility of p-type SnO2,” J. Mater. Chem., vol. 22, no. 48, pp. 25236–25245, Dec. 2012, doi: 10.1039/c2jm34352e. Crossref
P. Ágoston, K. Albe, R. M. Nieminen, and M. J. Puska, “Intrinsic n-type behavior in transparent conducting oxides: a comparative hybrid-functional study of In2O3, SnO2, and Zno,” Phys. Rev. Lett., vol. 103, no. 24, Dec. 2009, doi: 10.1103/PhysRevLett.103.245501. Crossref
M. Ferreira, J. Loureiro, A. Nogueira, A. Rodrigues, R. Martins, and I. Ferreira, “SnO2 thin film oxides produced by rf sputtering for transparent thermoelectric devices,” Mater. Today Proc., vol. 2, no. 2, pp. 647–653, 2015, doi: 10.1016/j.matpr.2015.05.090. Crossref
Q. Wang, L. Han, L. Wu, T. Zhang, S. Li, and P. Lu, “Strain effect on thermoelectric performance of inse monolayer,” Nanoscale Res. Lett., vol. 14, no. 1, Dec. 2019, doi: 10.1186/s11671-019-3113-9. Crossref
H. J. Goldsmid and J. W. Sharp, “Estimation of the thermal bandgap of a semiconductor from seebeck measurements,” J. Electron. Mater., vol. 28, pp. 869–872, Jul. 1999, doi: 10.1007/s11664-999-0211-y. Crossref
Article Metrics
Metrics powered by PLOS ALM
Refbacks
- There are currently no refbacks.
Copyright (c) 2022 National Research and Innovation Agency

This work is licensed under a Creative Commons Attribution-NonCommercial-ShareAlike 4.0 International License.