Thermopower Enhancement of Rutile-type SnO2 Nanocrystalline Using Facile Co-Precipitation Method
Abstract
Metal oxide semiconductor has attracted so much attention due to its high carrier mobility. Herein, thermoelectric study of nanocrystalline SnO2 through a simple co-precipitation method is conducted to enhance the Seebeck coefficient (S). X-ray diffraction, thermogravimetric analysis (TGA), resistivity (r), Seebeck coefficient (S), and power factor (PF) measurements are conducted to analyze the thermoelectric properties of the material. The measurements show that there are two interesting results, which are the unusual resistivity behavior and the high value of the S. Resistivity behavior shows a non-reflective intermediate semiconductor-metals behavior where the turning point occurs at 250 o C. This behavior is strongly correlated to the surface oxide reaction due to annealing temperature. The maximum S likely occurs at 250 ºC, since the curve shows a slight thermopower peak at 250 ºC. The value of the S is quite high with around twenty times higher than other publications about SnO2 thermoelectric material, this happens due to the bandgap broadening. The energy gap of SnO2 calculated using density functional theory (DFT), which was performed by Quantum Espresso 6.6. The result shows that there is a broadening energy gap at different momentum or wave factor. Nanocrystalline semiconductors material is giving an impact to increase the width of bandgap due to quantum confinement and could enhance the thermopower especially in SnO2 nanocrystalline
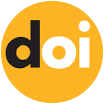
Keywords
References
Y. Yin, B. Tudu, and A. Tiwari, “Recent advances in oxide thermoelectric materials and modules,” Vacuum, vol. 146, pp. 356–374, 2017. Crossref
A. J. Minnich, M. S. Dresselhaus, Z. F. Ren, and G. Chen, “Bulk nanostructured thermoelectric materials: Current research and future prospects,” Energy Environ. Sci., vol. 2, no. 5, pp. 466–479, 2009. Crossref
G. S. Nolas, J. Sharp, and J. Goldsmid, Thermoelectrics: Basic Principles and New Materials Developments. New York: Springer-Verlag Berlin Heidelberg, 2013.
B. Poudel et al., “High-thermoelectric performance of nanostructured bismuth antimony telluride bulk alloys,” Sci., vol. 320, no. 1996, pp. 634–638, 2008. Crossref
S. A. Miller et al., “SnO as a potential oxide thermoelectric candidate,” J. Mater. Chem. C, vol. 5, no. 34, pp. 8854–8861, 2017. Crossref
C. Li, F. Jiang, C. Liu, P. Liu, and J. Xu, “Present and future thermoelectric materials toward wearable energy harvesting,” Appl. Mater. Today, vol. 15, pp. 543–557, 2019. Crossref
Y. Zheng et al., “Designing hybrid architectures for advanced thermoelectric materials,” Mater. Chem. Front., vol. 1, no. 12, pp. 2457–2473, 2017. Crossref
Z. G. Chen, G. Hana, L. Yanga, L. Cheng, and J. Zou, “Nanostructured thermoelectric materials: Current research and future challenge,” Prog. Nat. Sci. Mater. Int., vol. 22, no. 6, pp. 535–549, 2012. Crossref
J. F. Nakahara, T. Takeshita, M. J. Tschetter, B. J. Beaudry, and K. A. Gschneidner, “Thermoelectric properties of lanthanum sulfide with Sm, Eu, and Yb additives,” J. Appl. Phys., vol. 63, no. 7, pp. 2331–2336, 1988. Crossref
C. M. Bhandari and D. M. Rowe, “Silicon-germanium alloys as high-temperature thermoelectric materials,” Contemp. Phys., vol. 21, no. 3, pp. 219–242, 1980. Crossref
D. M. Rowe and V. S. Shukla, “The effect of phonon-grain boundary scattering on the lattice thermal conductivity and thermoelectric conversion efficiency of heavily doped fine-grained, hot-pressed silicon germanium alloy,” J. Appl. Phys., vol. 52, no. 12, pp. 7421–7426, 1981. Crossref
S. at Tanusilp and K. Kurosaki, “Si-based materials for thermoelectric applications,” Mater. (Basel)., vol. 12, no. 12, 2019. Crossref
B. C. Sales, D. Mandrus, and R. K. Williams, “Filled skutterudite antimonides: A new class of thermoelectric materials,” Science, vol. 272, no. 5266, pp. 1325–1328, 1996. Crossref
B. M. S. Dresselhaus et al., “New directions for low-dimensional thermoelectric materials,” Adv. Mater., vol. 19, pp. 1043–1053, 2007. Crossref
Y. Takagiwa, Y. Pei, G. Pomrehn, and G. J. Snyder, “Dopants effect on the band structure of PbTe thermoelectric material,” Appl. Phys. Lett., vol. 101, no. 9, pp. 1–4, 2012. Crossref
N. Okinaka and T. Akiyama, “Thermoelectric properties of nonstoichiometric TiO as a promising oxide material for high-temperature thermoelectric conversion,” in 24th Int. Conf. Thermoelectr., USA, 2005, pp. 34–37. Crossref
Y. Feng et al., “Metal oxides for thermoelectric power generation and beyond,” Adv. Compos. Hybrid Mater., vol. 1, no. 1, pp. 114–126, 2018. Crossref
A. Paulson, N. A. M. Sabeer, and P. P. Pradyumnan, “Enhanced thermoelectric property of oxygen deficient nickel doped SnO2 for high temperature application,” Mater. Res. Express, vol. 5, no. 4, pp. 1–21, 2018. Crossref
Z. Kerrami, A. Sibari, O. Mounkachi, A. Benyoussef, and M. Benaissa, “SnO2 improved thermoelectric properties under compressive strain,” Comput. Condens. Matter, vol. 18, 2019. Crossref
M. B. Sahana, C. Sudakar, A. Dixit, J. S. Thakur, R. Naik, and V. M. Naik, “Quantum confinement effects and band gap engineering of SnO2 nanocrystals in a MgO matrix,” Acta Mater., vol. 60, no. 3, pp. 1072–1078, 2012. Crossref
C. G. Fonstad and R. H. Rediker, “Electrical properties of high-quality stannic oxide crystals,” J. Appl. Phys., vol. 42, no. 7, pp. 2911–2918, 1971. Crossref
M. Ferreira, J. Loureiro, A. Nogueira, A. Rodrigues, R. Martins, and I. Ferreira, “SnO2 thin film oxides produced by rf sputtering for transparent thermoelectric devices,” Mater. Today Proc., vol. 2, no. 2, pp. 647–653, 2015. Crossref
M. M. Bagheri-Mohagheghi, N. Shahtahmasebi, M. R. Alinejad, A. Youssefi, and M. Shokooh-Saremi, “Fe-doped SnO2 transparent semi-conducting thin films deposited by spray pyrolysis technique: Thermoelectric and p-type conductivity properties,” Solid State Sci., vol. 11, no. 1, pp. 233–239, 2009. Crossref
Z. M. Gibbs et al., “Band gap estimation from temperature dependent Seebeck measurement - Deviations from the 2e|S|maxTmax relation," Appl. Phys. Lett., vol. 106, pp. 022112-1-022112–5, 2015. Crossref
A. Rajaeiyan and M. M. Bagheri-Mohagheghi, “Comparison of sol-gel and co-precipitation methods on the structural properties and phase transformation of γ and α-Al2O3 nanoparticles,” Adv. Manuf., vol. 1, no. 2, pp. 176–182, 2013. Crossref
V. D. Mote, Y. Purushotham, and B. N. Dole, “Williamson-Hall analysis in estimation of lattice strain in nanometer-sized ZnO particles,” J. Theor. Appl. Phys., vol. 6, pp. 1–8, 2012. Crossref
G. K. Williamson and W. H. Hall, “X-Ray broadening from filed aluminium and wolfram,” Acta Metall., vol. 1, pp. 22–31, 1953. Crossref
W. H. Baur, “Über die Verfeinerung der Kristallstrukturbestimmung einiger Vertreter des Rutiltyps: TiO2, SnO2, GeO2 und MgF2,” Acta Crystallogr., vol. 9, no. 6, pp. 515–520, 1956. Crossref
L. B. McCusker, R. B. Dreele, D. E. Cox, D. Louer, and P. Scardi, “Rietveld refinement guidelines,” J. Apply. Cryst., vol. 32, pp. 36–50, 1999.
N. S. Gonçalves, J. A. Carvalho, Z. M. Lima, and J. M. Sasaki, “Size-strain study of NiO nanoparticles by X-ray powder diffraction line broadening,” Mater. Lett., vol. 72, pp. 36–38, 2012. Crossref
M. Aziz, S. S. Abbas, and W. R. W. Baharom, “Size-controlled synthesis of SnO2 nanoparticles by sol-gel method,” Mater. Lett., vol. 91, pp. 31–34, 2013. Crossref
V. Kumar et al., “Effect of solvent on crystallographic, morphological and optical properties of SnO2 nanoparticles,” Mater. Res. Bull., vol. 85, pp. 202–208, 2017. Crossref
H. Uchiyama, H. Ohgi, and H. Imai, “Selective preparation of SnO2 and SnO crystals with controlled morphologies in an aqueous solution system,” Cryst. Growth Des., vol. 6, no. 9, pp. 2186–2190, 2006. Crossref
S. Loganathan, R. B. Valapa, R. K. Mishra, G. Pugazhenthi, and S. Thomas, "Thermogravimetric analysis for characterization of nanomaterials," in Thermal and Rheological Measurement Techniques for Nanomaterials Characterization, vol. 3., Netherlands: Elsevier Inc., 2017. Crossref
Interpreting TGA curves: information for users of Mettler Toledo thermal analysis systems,” Mettler Toledo, 2001, pp. 1–20.
J. Huang, N. Matsunaga, K. Shimanoe, N. Yamazoe, and T. Kunitake, “Nanotubular SnO2 templated by cellulose fibers: Synthesis and gas sensing,” Chem. Mater., vol. 17, no. 13, pp. 3513–3518, 2005. Crossref
V. Nadutov, A. Perekos, V. Kokorin, S. Konoplyuk, and T. Kabantsev, “Influence of oxidation on electrical properties of compacted Cu nanopowders,” Nanoscale Res. Lett., vol. 11, pp. 1-4, 2016. Crossref
M. Markov, S. E. Rezaei, S. N. Sadeghi, K. Esfarjani, and M. Zebarjadi, “Thermoelectric properties of semimetals,” Phys. Rev. Mater., vol. 3, no. 9, pp. 1–7, 2019. Crossref
H. J. Goldsmid and J. W. Sharp, “Estimation of the thermal band gap of a semiconductor from Seebeck measurements,” J. Electron. Mater., vol. 28, no. 7, pp. 869–872, 1999. Crossref
P. Giannozi et al., “Advanced capabilities for materials modelling with Quantum ESPRESSO,” J. Phys. Condens. Matter, vol. 29, no. 46, pp. 1–30, 2017.
P. E. Blöchl, “Projector augmented-wave method,” Phys. Rev. B, vol. 50, no. 24, pp. 17953–17979, 1994.
J. P. Perdew et al., “Restoring the density-gradient expansion for exchange in solids and surfaces,” Phys. Rev. Lett., vol. 100, no. 13, pp. 1–4, 2008. Crossref
Á. Morales-García, R. Valero, and F. Illas, “An empirical, yet practical way to predict the band gap in solids by using density functional band structure calculations,” J. Phys. Chem. C, vol. 121, no. 34, pp. 18862–18866, 2017. Crossref
W. Zhou, Y. Liu, Y. Yang, and P. Wu, “Band gap engineering of SnO2 by epitaxial strain: Experimental and theoretical investigations,” J. Phys. Chem. C, vol. 118, pp. 6448–6453, 2014. Crossref
Y. Zhao and J. Zhang, “Microstrain and grain-size analysis from diffraction peak width and graphical derivation of high-pressure thermomechanics,” J. Appl. Cryst., vol. 41, no. 6, pp. 1095–1108, 2008. Crossref
M. B. Sahana et al., “Bandgap engineering by tuning particle size and crystallinity of SnO2 - Fe2O3 nanocrystalline composite thin films,” Appl. Phys. Lett., vol. 93, no. 23, pp. 2–5, 2008. Crossref
S. Hao, V. P. Dravid, M. G. Kanatzidis, and C. Wolverton, “Computational strategies for design and discovery of nanostructured thermoelectrics,” Nature Partner J. Comput. Mater., vol. 5, pp. 1–10, 2019. Crossref
Article Metrics
Metrics powered by PLOS ALM
Refbacks
- There are currently no refbacks.
Copyright (c) 2020 National Research and Innovation Agency

This work is licensed under a Creative Commons Attribution-NonCommercial-ShareAlike 4.0 International License.